All published articles of this journal are available on ScienceDirect.
Effects of Trunk Functional Capacity on the Control of Angular Momentum During Manual Wheelchair Braking
Abstract
Background:
Manual wheelchair braking induces an upper body angular impulse which must be controlled by joint moments to prevent a forward fall.
Objective:
The purpose of this study was to determine the effects of trunk functional capacity (low, high) on the rate of change in sagittal plane upper body angular momentum during manual wheelchair braking.
Methods:
Eight wheelchair users (4 low function, 4 high function) completed 10 trials of abrupt wheelchair braking. Trunk segment angles and abdominal joint angles, and normalized upper-body angular impulses were computed for each trial. Linear mixed effects models with initial velocity as a covariate were used to determine differences between groups.
Results:
The low function group had a higher angular impulse than the high function group with a mean difference (MD) ± SE of 1.59 ± 0.65 N∙m∙s/kg/m2, with a 95% CI 0.287 to 2.89, p = 0.018. The low function group also had a higher trunk segment extension angle, MD = –13.98 degrees, 95% CI: –26.27 to –1.69, p = .027. The low function group employed a greater trunk segment range of motion during braking when compared to the high function group, MD = 12.14 degrees, 95% CI: –24.48 to 0.21, p = 0.054. Lower trunk functional capacity wheelchair users had a higher fall risk during braking.
Conclusion:
Wheelchair users with less trunk function may be at increased risk of suffering a fall when bringing their wheelchair to an abrupt stop due to an impaired ability to arrest angular momentum.
1. INTRODUCTION
Manual wheelchair braking is an important skill for wheelchair users. This skill is utilized in wheelchair sports [1-3] as well as casual locomotion, with wheelchair users engaging in up to 250 start/stop activities per day [4]. As critical as this skill is to wheelchair users, very little research has been conducted on this aspect of wheelchair mobility, particularly with respect to functional capacity.
Seated balance is impaired in wheelchair users across a functional spectrum [5], and this is demonstrated by the high incidence of falls in this population. In one study of 659 wheelchair users, 31% reported a total of 553 fall events, with 15% of these occurring during wheelchair propulsion [6]. The main cause of falls in this population was found to be loss of balance during functional activities [7]. Manual wheelchair braking uniquely challenges balance control systems in wheelchair users and requires a coordination between the upper limb joints and body segments to decelerate the wheelchair while resisting the forward momentum of the wheelchair/user system, as well as the momentum of the upper body once the wheelchair has come to a stop. Wheelchair users have been shown to have an impaired ability to maintain balance both in stable environments and in response to external perturbations, with differences existing across a functional spectrum. Kamper et al. [8] used a tilting platform to simulate deceleration conditions similar to those seen in a moving vehicle to examine the relationship between limits of stability and balance in response to external perturbations in the sagittal plane, and found that cervical spinal cord injured subjects lost balance at a smaller magnitude of disturbance than thoracic spinal cord injured subjects. These results are consistent with functional differences seen in seated balance and functional reach of wheelchair users and demonstrate the role of trunk function in maintaining balance. These difficulties in balance control are influenced both by lack of control of postural muscles as well as impairments in sensorimotor integration of the trunk and lower body [9]. Given the inertial forces that wheelchair users are exposed to during propulsion, particularly in the anteroposterior direction [10], similar challenges could be expected when a wheelchair user uses their hands as friction brakes [11].
Changes in velocity during wheelchair locomotion (both propulsion and braking activities) result in changes in upper body angular momentum that must be controlled by joint moments to prevent a loss of balance. The trunk is the largest body segment and is the largest contributor to angular acceleration during locomotion [12], so the ability to control trunk acceleration is critical to maintaining balance. Cooper et al. [13] examined the effects of different braking methods on fall risk in an electric power wheelchair using a crash test dummy. They reported that gradual braking (−3.0 m/s2 to −3.3 m/s2) caused angular accelerations of the upper body of 746 d/s2 to 1409 d/s2. The combination of increased velocity prior to braking and abrupt braking (−5.6 m/s2 to −6.3 m/s2) resulted in upper body angular accelerations of 1476 d/s2 to 1883 d/s2, and a higher percentage of forward falls from the wheelchair. Cooper et al. [13], recommended that wheelchair users with less trunk control utilize a braking strategy that allows them to come to a more gradual stop. Unfortunately, there are occasions where the manual wheelchair users must brake abruptly to avoid collisions, such as turning a blind corner on a sidewalk.
The role of trunk function and the importance of a better understanding of how wheelchair users stop their wheelchairs are interrelated. Gu et al. [14] put it succinctly, stating “quantification requires knowledge not only of how much angular momentum must be arrested, but also what moment can be developed to do that arresting.” Functional capacity of the trunk influences both propulsion [15] and deceleration/braking activities [16], and the degree to which a wheelchair user has the ability to actively engage their trunk to push or stop their wheelchair is influenced by their limits of stability. A wheelchair user can only engage in trunk flexion to generate power during propulsion to the extent that they do not exceed their anterior limits of stability. Conversely, a wheelchair user can only engage in trunk extension to prevent themselves from falling forward to the extent that they do not exceed their posterior limit of stability. While trunk extension has been observed during ramp descent, the role of functional capacity in abrupt braking remains to be seen. Given the role of braking in wheelchair mobility, there remains a need for more information on how manual wheelchair users can avoid forward falls during abrupt braking.
Therefore, the purpose of this study was to determine the effects of trunk functional capacity (low, high) on the rate of change in sagittal plane upper body angular momentum during manual wheelchair braking.
2. MATERIALS AND METHODS
2.1. Participants
Eight wheelchair users were recruited to complete this study (4 men, 4 women). Mean age of wheelchair users was 24.75 ± 7.57 years, and mass was 65.10 ± 14.15 kg. All participants were collegiate wheelchair basketball players and placed into groups based on their functional classification as determined by trained classifiers with the National Wheelchair Basketball Association. This was done because there were not enough participants in each classification to conduct analyses. This method of stratification has been used similarly by Wilson et al. [17]. Functional Classifications ranged from 1.0 to 3.5. Self-reported shoulder pain or injury within the last six months was considered an exclusion criterion. Use of a wheelchair as primary means of locomotion were required to participate in this study. Participants were divided into two subgroups of 4 each: high functional classification (HFC), in which the participant’s functional classification was 3.0 or above, and low functional classification (LFC), in which the participant’s functional classification was 2.5 or below [17].
2.2. Experimental Design, Sample Precision and Sensitivity
This observational study was designed to take advantage of the enhanced statistical power of repeated measures designs by using trials as a covariate as opposed to averaging trials. Sample size, sample power and sample precision were estimated using a data simulation [18] with 4 subjects per group (low, high) and 10 trials per subject. SAS GLIMMIX was used to fit random intercepts for each subject with trials as a covariate and an AR(1) variance-covariance matrix.
Our sensitivity simulation used a meaningful significant difference in normalized angular impulse of 1.0 ± 0.42 N∙m∙s/kg/m2 (mean ± se) with a 95% CI of 0.27 to 1.72. Estimated power was computed using a between subject variance of 1.3, within subject variance of 0.33 and a correlation between trials of 0.54. Using these parameters as input our design was estimated to detect mean differences in normalized angular impulse of 0.5 N∙m∙s/kg/m2 and 1.0 N∙m∙s/kg/m2 with a power of 0.27, 0.77, respectively.
2.3. Experimental Procedures
Data collection took place during a single visit to the Biomechanics Laboratory. Participants used their personal wheelchair that they used for everyday use to minimize differences in wheelchair configuration. Reflective markers (14 mm) were attached bilaterally to the skin over anatomical landmarks. Acromion process (RAC, LAC), joint center of the shoulder complex (RADL, RPDL, LADL, LPDL), neck in line with C7 (RNECK, LNECK), C7, T8, T2, L1, L3, L5 vertebrae, superior most point of iliac crest in the sagittal plane (RPP, LPP), anterior superior iliac spine (RAS, LAS), posterior superior iliac spine (RPS, LPS), greater trochanters (RHP, LHP), medial and lateral epicondyles of the femur (RMK, RLK, LMK, LLK), medial and lateral epicondyle of the humerus (RMEL, RLEL, LLEL, LMEL), radial and ulnar epicondyles (RWRR, RWRU, LWRR, LWRU), second third, and fifth metacarpals (LHR, LHM, LHU, RHR, RHM, RHU) medial and lateral malleoli (RMA, RLA, LMA, LLA), first metatarsal, base and fifth of the metatarsals. Markers were also placed on the top of the head (THEAD), forehead (AHEAD), occipital bone (PHEAD), zygomatic bone (RHEAD, LHEAD). Non-collinear markers on molded thermo-plastic shells were placed on the posterior thorax, upper arms, forearms, proximal thighs, and distal shanks. Three tracking markers were placed on the medial, lateral, and posterior heel. All anatomical markers were then removed for wheelchair braking trials.
Prior to the collection of the braking trials, subjects had the opportunity to practice bringing their wheelchair to an abrupt stop at a predefined location within the laboratory. Participants were then asked to complete ten trials where they were instructed to push their wheelchairs as quickly as possible to a predefined spot on the floor of the laboratory, covering a distance of approximately ten meters, and then bring their wheelchair to an abrupt stop as quickly as possible. The number of pushes taken to reach this spot totaled 4 to 5 propulsion cycles. Trials were disqualified if the participant lost their balance, and the trial was repeated.
2.4. Data Analysis
Visual 3D (C-Motion, Germantown, MD, USA) was used to process three-dimensional kinematic and kinetic data for each participant. Marker trajectories were filtered with a fourth order recursive Butterworth low-pass filter with cutoff frequency of 6 Hz.
Kinematic data were low-pass filtered using fourth-order Butterworth filters with cut-off frequencies of 6 Hz. A 14-segment model including the head, torso, pelvis, upper arms, lower arms, thighs, shanks and feet was used to determine the COM location and velocity of each segment. Segment masses and inertial properties were determined using de Leva [19] (1996). Upper-body angular momentum (head, torso, pelvis, arms and hand) about the medial/lateral axis of the upper body center of mass was determined using the following equation:
![]() |
where are the position, velocity and angular velocity vectors of the ith segment’s CM,
, are the position and velocity vectors of the upper body CM, mi and Ii are the mass and moment of inertia of the ith segment and n is the number of segments. Angular momentum (H) was normalized by dividing by the mass of the upper body segments (kg) and upper body height squared. The upper body height was defined as the distance from the hip joint to top of head (m). The direction of the angular momentum vector was defined by the right hand rule with positive angular momentum defining rotation of the wheelchair user toward the back of the wheelchair and negative angular momentum defining the magnitude of rotation causing or tending to cause the user to fall forward out of the wheelchair. Angular impulse was computed by integrating the negative angular momentum. The negative angular impulse quantifies the amount of momentum that must be resisted by joint moments to prevent a forward fall of the user from wheelchair.
2.5. Statistical Analysis
Separate SAS version 9.4 proc GLIMMIX linear mixed effects models were used to compare differences in each dependent variable (trunk segment angles and angular impulse) with functional capacity as a fixed effect grouping factor (high, low), subjects as a random factor with trials and initial horizontal velocity as a covariate. Separate intercepts were fit for each subject using an unstructured variance-covariance matrix to account for the correlations between trials. A significant main effect for group was followed by post hoc analysis with Tukey correction for multiple comparisons between groups with alpha set at 0.05. Outcome data were reported as mean differences, standard error of the difference, 95% confidence intervals and Cohen's d effect sizes.
3. RESULTS
3.1. Initial Horizontal Velocity prior to Braking
There were no differences between groups (high, low) in the initial horizontal velocity of the wheelchair-user prior to the initiation of abrupt wheelchair braking, mean difference MD ± SE, MD = 0.17 ± 0.24 m/s, with a 95% CI of -0.31 to 0.64, p = 0.49, Cohen’s d = 0.11. The high function group had an initial horizontal velocity of 3.02 ± 0.17 m/s, CI: 2.68 to 3.36 and the low function group had an initial horizontal velocity of 2.85 ± 0.17 m/s, CI: 2.51 to 3.19.
3.2. Upper Body Kinematics
There was a significant difference between groups for trunk segment extension, mean difference MD ± SE, MD = 13.98 ± 6.15 degrees, with a 95% CI 1.69 to 26.27, p = 0.027, Cohen’s d = 0.359. The low functional classification group leaned their trunk back further at the onset of braking their wheelchairs than the high functional classification group (30.32 ± 4.35 degrees, 95% CI of 21.63 to 39.02, and 16.34 ± 4.35 degrees, 95% CI of 7.66 to 25.03, respectively).
There were no significant differences between groups for the trunk segment flexion attained at the end of braking, mean difference MD ± SE, MD = 1.84 ± 8.28 degrees, with a 95% CI −18.39 to 14.71, p = 0.825, Cohen’s d = 0.035. In the low functional group subjects had a final trunk segment flexion angle of −0.02 ± 5.85 degrees with a 95% CI of −11.72 to 11.68. Final trunk segment flexion angle in the high function group was -1.86 ± 5.85 degrees with a 95% CI of −13.56 to 9.83.
There were no significant differences between groups in the trunk segment total range of motion, mean difference MD ± SE, MD = 12.13 ± 6.18 degrees, with a 95% CI −24.48 to 0.21, p = 0.054, Cohen’s d = 0.311. The high function group had a mean ± SE trunk segment range of motion of 18.21 ± 4.36 degrees, 95% CI of 9.48 to 26.93 to bring the upper body momentum to rest. Subjects in the low function group exhibited a trunk segment range of motion of 30.34 ± 4.36 degrees, 95% CI of 21.61 to 39.08 to bring the upper body momentum to rest.
3.3. Normalized Upper-body Angular Momentum and Angular Impulse
A typical trial of normalized upper body angular momentum about a medial/lateral axis of the upper body CM during abrupt wheelchair braking is shown in Fig. (1). During the initial phase of braking the angular momentum is positive as a result of the wheelchair user leaning backward (t = 0 to t = 0.5 s). From t = 0.5 to t = 2.0 s upper body angular momentum is negative as the wheelchair decelerates causing negative angular momentum.
There was a significant difference in the normalized upper-body angular impulse about a medial/lateral axis of the upper-body center of mass between the groups, mean difference MD ± SE, MD = 1.59 ± 0.65 N∙m∙s/kg/m2, with a 95% CI 0.287 to 2.89, p = 0.018, Cohen’s d = 0.386. Note, angular impulses were multiplied by 100 to improve significant digits for statistical analysis. Subjects in the low function group had greater fall inducing normalized upper-body angular impulse with −3.87 ± 0.46 N∙m∙s/kg/m2, 95% CI of −2.96 to −4.79. In the high function group, the fall inducing angular impulse was with −3.87 ± 0.46 N∙m∙s/kg/m2, 95% CI of −1.37 to −3.20.
4. DISCUSSION
Abrupt manual wheelchair braking generates upper body angular impulse which must be counteracted by joint moments to prevent a forward fall of the user from the wheelchair. The upper limits of this counteracting torque is determined by neuromuscular control of the trunk and lower limbs by the wheelchair user. During the initial phase of braking the wheelchair user leans the trunk backward effectively increasing the range of motion over which he/she can counteract the angular impulse induce by wheelchair braking. Subjects in our low functional classification leaned their trunk backwards 13.97 degrees more than subjects in our high functional classification group. The low function group also utilized a larger range of motion of 12.14 degrees to bring the upper body momentum to rest during braking. These differences in trunk segment angular kinematics during braking directly reflect the functional capacity of the wheelchair user. High functional capacity subjects can generate greater extension torque and therefore are able to control the forward fall inducing angular impulse over a smaller trunk range of motion.
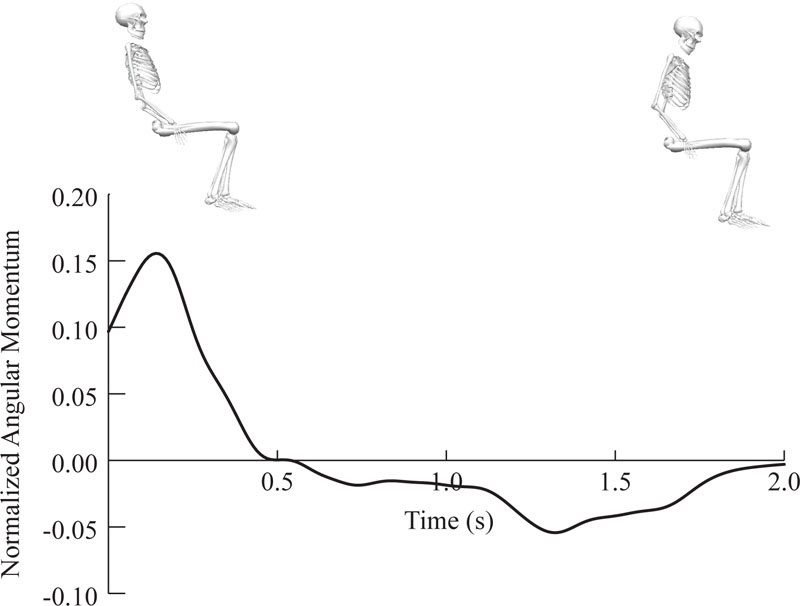
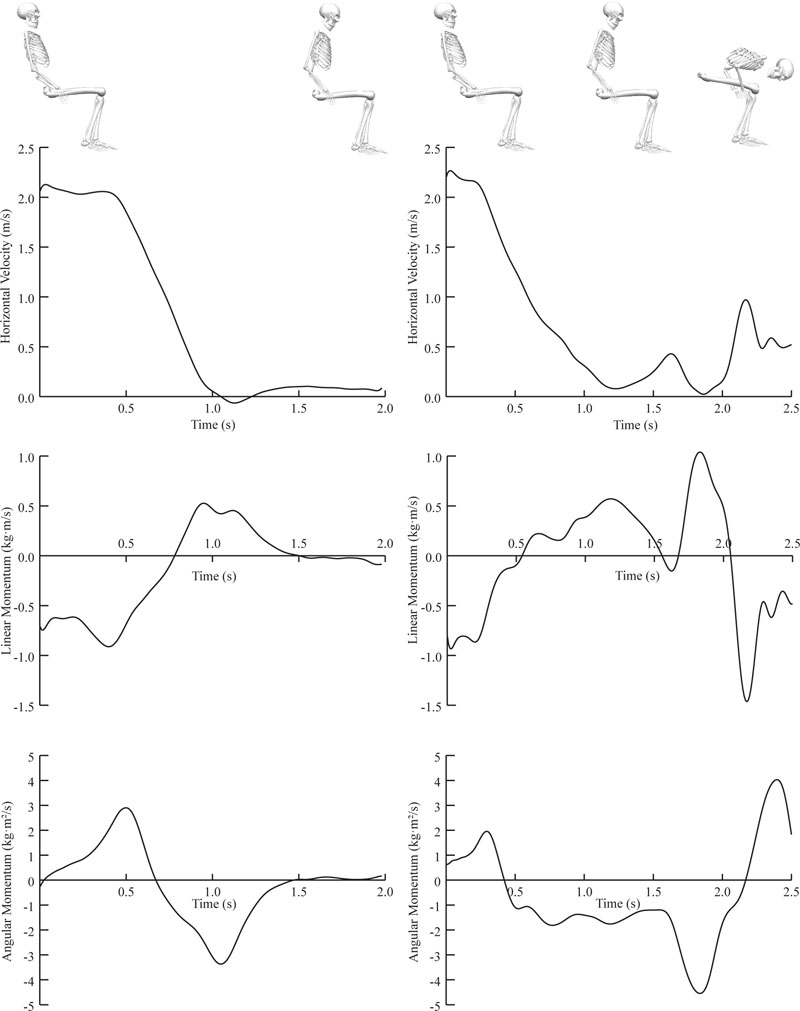
To successfully prevent a forward fall from the wheelchair during abrupt braking the wheelchair user must attenuate forward angular momentum as the wheelchair decelerates by applying a trunk extension joint moment. Fig. (2) illustrates an example of successful (left) and unsuccessful braking (right) for a single subject. The velocity, normalized relative linear momentum of the upper body, and normalized angular momentum is shown for each trial. Graphs in the middle row depict the linear momentum of the upper body relative to the wheelchair velocity. In successful braking on the left, initial relative linear momentum is negative due to trunk extension, from 0.75 s to 1.5 s the upper body linear momentum continues to move forward as the chair decelerates to 0 m/s (top). The angular momentum graph on the bottom left illustrates that the subject successfully attenuates the negative angular momentum caused by deceleration of the wheelchair, thus preventing a forward fall. In the fall condition shown on the right, the subject did not have the functional capacity to arrest the angular momentum of their trunk. Sensing the impending loss of balance at 1.2 s the subject releases the pushrims, causing chair/user velocity increase from 1.2 to 1.7 s. The subject then grabs the vertical uprights of the wheelchair to prevent a forward fall.
As mentioned, there is evidence to suggest that trunk function plays a role in the anticipatory response to changes in position to maintain balance [20-22], including activation of the abdominal and trunk extensor muscles. In this study the low function group had a higher normalized angular impulse than the higher function group, suggesting that they have a decreased ability to control the forward momentum of the trunk upon bringing the wheelchair to a complete stop. Coupled with a smaller anterior stability limit, this puts users with less functional control of their trunk at an increased risk of falling out of their wheelchair if angular impulse exceeds the moment that can be developed to arrest it before the center of mass falls outside the functional base of support. Gait termination studies provide a unique insight into this phenomenon. During transition periods such as gait termination (or wheelchair braking) the CNS is uniquely challenged to respond to changes to the environment, and must determine factors such as appropriate braking forces, including the direction and magnitude of those forces, to maintain to maintain the center of mass over the functional base of support [23]. Combining an increased angular impulse with a decreased ability to control the center of mass (which, for these purposes consists of the upper body) puts the user at an increased risk of suffering a fall. This has been demonstrated in able-bodied subjects with decreased proprioception in type 2 DM [23], incomplete spinal cord injury [24], and Parkinson’s Disease [25]. One factor that may influence this in wheelchair users is decreased proprioception in individuals with lower functional capacity. In a study examining wheelie performance in wheelchair users, Kauzlarich & Thacker [26] determined that proprioception, vision, and vestibular function may be as important in the balance of a wheelie as they are in maintaining standing balance. It is possible that decreased proprioception of the lower body and trunk in wheelchair users with lower functional capacity may hinder their ability to sense and respond to perturbations which may lead to loss of balance. Our results provide insight on the role that functional capacity in manual wheelchair users plays in maintaining balance in manual wheelchair braking.
Very little research has been conducted on trunk utilization and movement patterns during manual wheelchair braking. In a study examining different braking strategies of electric power wheelchairs Dvorznak et al. [27] observed that when across three braking conditions, the center of gravity and height of the wheelchair directly influenced the tipping moment of the wheelchair and user. If this moment is great enough, this can result in the wheelchair tipping forward. Alternatively, if the center of gravity is higher, the user may fall out of the wheelchair. Dvorznak et al. [27] did not study the effects functional capacity or the user’s ability to control prevent the falls. Additionally, this research was conducted in electric power wheelchairs, so extrapolating the results to manual wheelchair users may be of limited utility. In a study examining the response to motor vehicle braking, Kamper et al. [8], found that SCI subjects lost balance at thresholds below those seen in standard braking patterns in motor vehicles. These subjects were not able to use their hands or arms to support themselves, and the inertia they were required to resist was the result of a motor vehicle, not wheelchair propulsion. We recommend that future studies focus further on the strategies that wheelchair users with lower functional capacity may use to mitigate the risk of falling during abrupt braking.
Additionally, inferences can be made from work that has been done regarding the role of the trunk during forward propulsion. For example, Rice et al. [28] found that the trunk actually moved backwards at the beginning of the push phase and attributed this movement to the reactive forces of the pushrim in individuals with impaired trunk control. Taken with the results from the present study, we hypothesize that manual wheelchair users with lower functional capacity may have preemptively leaned their trunk backwards in order to try to prevent their trunk from falling forward once their hands came into resistance from the forward momentum of the handrim. This increases the braking distance, decreasing the overall force incurred on the trunk. This is consistent with work conducted by Yang et al. [22], who demonstrated that able-bodied volunteers who propelled a manual wheelchair showed activation of the trunk muscles even before the initiation of the push cycle, stabilizing their trunk in anticipation of the reactionary forces incurred during the push itself.
Subjects in our lower functional classification group compensated for their inability to generate a trunk extension moment during wheelchair braking by employing more trunk extension prior to braking. This increased the amount of distance that their center of mass needed to travel before reaching their anterior limit of stability. There is evidence to suggest that backrest height influences several outcomes with respect to wheelchair propulsion biomechanics. Yang et al. [29] found that wheelchair users with a lower backrest had a greater range of shoulder motion, increased stroke angle, and reduced cadence during forward propulsion, all of which decreased the risk of injury to the shoulder complex. According to the IWBF Player Classification Commission [30], lower classification players rely on backrest height for improved stability, so future work should examine the role of backrest height in trunk kinematics during braking.
4.1. Limitations
We used reflective markers on the wheelchair to compute the velocity and acceleration of the chair and therefore determine the onset of braking. Instrumenting the wheels with either strain gauges or a torque sensor may give a more accurate measure of when braking was initiated. Additionally, we did not take note of the wheel diameters for each subject. There is evidence to suggest that wheel size may play a role in physiologic and biomechanical outcomes [31], and documenting that aspect may have provided insight into the biomechanics of wheelchair braking.
4.2. Future Directions
Future research should focus on the role of different wheelchair configurations in attenuating forces of manual wheelchair braking. There is evidence to suggest that manipulating the angle of the base of support may increase stability during propulsion [32], and this may extend to braking activities as well. Additionally, future research should examine the kinetics both at the pushrim and at the joints of the upper limb during abrupt wheelchair braking. This can be done using technology such as the SmartWHEEL (Out-Front, Pasco, WA). Future research should also focus on the relationship between reaction time and functional capacity, as those with less functional capacity may require a greater braking distance in order to maintain postural control and avoid falling out of the wheelchair. In gait termination research using ambulatory subjects, there is evidence to suggest that subjects may use a trunk flexion strategy to avoid falling [33], so the role of the trunk in wheelchair users with decreased functional capacity as a function of reaction time, particularly during activities of daily living that require abrupt stopping, may be of interest. The role of manual wheelchair braking in shoulder pain is also an area that should be further explored. Yildirim & Ozengin [34] found that individuals with less trunk control reported greater instances of shoulder pain on the Wheelchair User’s Shoulder Pain Index (WUSPI). Kinetic variables influencing shoulder, elbow, and wrist pain during braking would provide valuable information on the role of this aspect of wheelchair locomotion on the risk of injury across the functional spectrum.
In this investigation, due to the short nature of the braking trials, fatigue was not an issue. However, there are circumstances where fatigue may affect the wheelchair user’s ability to utilize their trunk to stop their wheelchair. Examples may include descending a long ramp, the period after competing in wheelchair sport, or at the end of a long day where the wheelchair user was actively pushing their wheelchair at a moderate intensity. Future investigations should incorporate these variables to examine the role of fatigue in wheelchair braking ability.
CONCLUSION
Functional capacity of the lower trunk affects the wheelchair user’s ability to generate sufficient trunk extension moments to control the rate of change in angular momentum during manual wheelchair braking. Lower functional capacity wheelchair users exhibited higher angular impulses and therefore greater fall risk during braking when compared to individuals with higher functional trunk capacity. These findings have significant implications of both wheelchair users and rehabilitation specialists who work with newly injured patients who will be required to use a wheelchair. These differences in functional capacity and their effect on wheelchair braking may require nuanced approaches to teaching wheelchair skills to new wheelchair users.
LIST OF ABBREVIATIONS
MD | = Mean Difference |
HFC | = High Functional Classification |
LFC | = Low Functional Classification |
ETHICS APPROVAL AND CONSENT TO PARTICIPATE
The study was approved by the Human Subjects Institutional Review Board at the University of Texas Arlington.
HUMAN AND ANIMAL RIGHTS
No animals were used in this study. Reported experiments on humans were in accordance with the Helsinki Declaration of 1975, as revised in 2008.
CONSENT FOR PUBLICATION
Participants were informed about study procedures and its possible benefits and risks and signed written informed consent.
STANDARDS OF REPORTING
STROBE guidelines were followed.
AVAILABILITY OF DATA AND MATERIALS
The data supporting the findings of the article are available at the following Zendo Repository: Effects of trunk functional capacity on the control of angular momentum during manual wheelchair braking | Zenodo md5:cc40d360ec57a8f6b684f810e71dd25c.
FUNDING
None.
CONFLICT OF INTEREST
The authors declare no conflict of interest, financial or otherwise.
ACKNOWLEDGEMENTS
We gratefully acknowledge Logan A. Ruhde for his assistance with data collection.